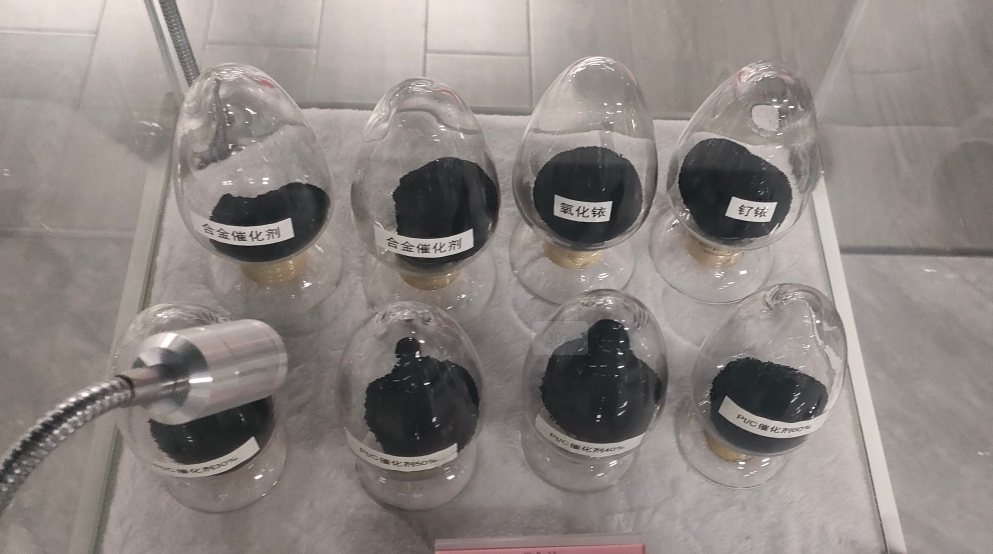
触媒の非活性化は水電気分解の重要な挑戦を、直接水素の生産の効率に影響を与えます。 電解槽触媒は、水素と酸素に水を分割する反応を駆動します。 しかし、これらの材料は、過酷な動作条件で劣化することが多いため、時間の経過とともに活動を減らすことができます。 この脆弱性を理解することは、電解質の耐久性と性能を向上させるために不可欠です.
要点
- 触媒の非活性化 水電解では主に水素の生産の効率を著しく減らすことができる加硫、毒および熱分解によって引き起こされます.
- 電解質純度の定期的なモニタリングと維持 安定した作動条件 触媒劣化を最小限に抑え、寿命を延ばすための重要な戦略です.
- 分光および電気化学的なテストのような高度の診断技術の実装は、触媒の劣化の早期検出を可能にし、タイムリーな維持および最適化を可能にします.
電解槽触媒活性化のメカニズム
ファーリングと表面ブロック
触媒表面に不必要な材料が蓄積すると、溶着が発生します。 これらの材料、多くの場合、反応副産物や不純物、ブロック活性部位、電解触媒の効率性を低下させます。 表面ブロックは触媒と反応剤間の相互作用を制限し、電気化学反応を妨げます。 時間が経つにつれて、このビルドアップは、触媒の洗浄または交換を必要とする、不可逆になることができます.
触媒中毒
特定の化学種が活性部位に強く結合したときに触媒中毒が起こります。 硫黄や塩素化合物などのこれらの種は、電解物や飼料の不純物から由来します。 中毒は、利用可能な活性部位の数を減らし、触媒作用の低下につながります。 中毒の重症度は、毒の種類と動作条件によって異なります.
熱分解
高温は電解槽触媒の構造を分解できます。 熱への長期暴露は焼結を引き起こします、触媒粒子が凝集し、表面面積を削減します。 このプロセスは、触媒の反応を容易にする能力を減少させます。 熱分解は熱管理の悪いシステムで特に問題です.
構造および形態学的変化
触媒は、機械的ストレスや化学反応による動作中に構造的変化を受けます。 これらの変化は、触媒の形態を変え、その性能に影響を与える。 たとえば、クラックや無効が形成され、アクティブな表面領域を減らすことができます。 そのような変化は、繰り返された循環または過酷な動作条件に起因することが多いです.
現場の解散とメタルリーチ
活性金属成分が電解液に溶解したときにサイト溶解が発生します。 腐食性の環境によって引き起こされるこの現象は、触媒材料の損失につながります。 メタルリーチは、電解触媒の全体的な活性を低減し、電解液を汚染し、プロセスをさらに適合させます.
触媒の活性化に寄与する要因
化学影響(例、不純物、反応副産物)
電解液または飼料の化学不純物は、電解槽触媒の性能に著しく影響を及ぼします。 塩化物イオンや硫黄化合物などのこれらの不純物は、触媒表面と相互作用し、汚染や中毒につながる。 酸素の基質か酸化金属を含む反応副産物はまた触媒で、活動的な表面区域を減らします.
注: 汚染物質の微量でも触媒分解を加速できます。 電解液純度の定期的なモニタリングは、これらの効果を最小限にするために不可欠です.
また、電解槽内の化学環境は、不要な副作用を促進することができます。 これらの反応は、活性部位をブロックしたり、触媒の化学組成物を変更したりする種を生成し、その効率性を低下させます.
電解質触媒の構造的特性
触媒の構造的特徴は、その耐久性と非活性化に対する抵抗を決定します。 高い気孔率および大きい表面区域が付いている触媒は頻繁によりよい初期性能を示します。 しかし、これらの特徴は、過酷な条件下で汚い、焼結、または構造崩壊により敏感にすることができます.
触媒組成も重要な役割を果たします。 特に腐食性の環境の酸化か分解のより速く、分解する材料の傾向。 例えば、非貴金属を含む触媒を電解液に浸し、長期的安定性を抑えることがあります.
動作条件(温度、電流密度、腐食性環境など)
作動条件は直接電解槽触媒の寿命に影響を与えます。 高温は熱分解および焼結を加速しま、過度の現在の密度は触媒の機械圧力を高めます。 酸性かアルカリの電解物、exacerbateの金属のleachingおよび場所のdissolutionのそれらのような腐食性の環境、.
ヒント 安定した動作条件を維持することで、触媒寿命を大幅に延ばすことができます。 極端な温度変動を避け、電流密度を最適化することは効果的な戦略です.
運用状態間の頻繁なサイクリングも構造疲労に貢献します。 このサイクリングは、触媒のアクティブ・サーフェスエリアと全体的な効率を削減し、クラックや空隙を引き起こします.
診断および特徴化の技術
触媒の非活性化の原因を理解するには、正確な診断と特徴化方法が必要です。 これらの技術は、研究者が劣化メカニズムを特定し、電解触媒の性能を評価するのに役立ちます.
分光器 メソッド
分光技術は、触媒の化学組成と表面特性に価値のある洞察を提供します。 X線光電子分光法(XPS)やラマン分光法などの手法は、酸化状態、化学結合、表面汚染物質の変化を検知します。 これらのツールは、研究者が触媒とその環境との相互作用を監視することができます。 たとえば、XPSは、飢餓や中毒に貢献する不純物の存在を明らかにすることができます.
顕微鏡解析
顕微鏡法は触媒の構造的および形態学的変化を調べます。 電子顕微鏡検査(SEM)および伝達電子顕微鏡検査(TEM)をスキャンすると、触媒表面の高解像度画像が提供されます。 これらの技術は熱か機械圧力によって引き起こされるひび、空隙、または粒子の凝集を識別します。 Atomic力顕微鏡検査(AFM)は、表面粗さや劣化パターンの詳細な解析を可能にし、三次元表面プロファイルを提供します.
電気化学テスト
電気化学技術は、動作条件下で電解槽触媒の活性と安定性を評価します。 円筒ボタムトリー(CV)および電気化学的インピーダンス分光法(EIS)は、反応キネシスやチャージトランスファー抵抗などの重要なパラメータを測定します。 これらの方法は、活性部位の喪失を定量化し、触媒性能に対する予防または中毒の影響を評価するのに役立ちます.
メタルリーチ検出のための高度な分析ツール
金属のleachingの検出は電解物の分解された金属のイオンを測定する高度用具を要求します。 誘導結合プラズマ質量分析法(ICP-MS)と原子吸収分光法(AAS)は、漏れた金属を正確に定量化します。 これらの方法は、サイト分解の程度と触媒分解への貢献を識別するのに役立ちます。 これらのツールを他の技術と組み合わせることで、触媒性能の包括的な理解が得られます.
電解質触媒の非活性化のための緩和戦略
触媒材料の設計および保護コーティング
耐久性を高めた触媒の設計は、大幅に非活性化を減らすことができます。 研究者は、汚染、中毒、熱劣化に対する高い耐性を持つ材料の開発に注力しています。 例えば、異動金属で貴金属を合金にすることで、触媒作用を維持しながら安定性が向上します。 酸化薄い層などの保護コーティング、腐食性環境から触媒をシールドします。 これらのコーティングは、触媒および有害物質間の直接接触を防ぎ、運用寿命を延ばします.
ヒント 低解散率の材料の選択は長期適用のよりよい性能を保障します.
運用の最適化と制御
動作条件の最適化により、電解触媒のストレスを最小限に抑えます。 安定した温度を維持し、現在の密度は熱分解および構造損傷の危険を減らします。 自動化された制御システムはキー変数を監察し、一貫した性能を保障します。 これらのシステムはまた、非活性化の早期兆候を検出し、オペレータは迅速に是正措置を取ることを可能にします.
注: 作動条件の極端な変動を避けることは、触媒への不可逆的な損傷を防ぐことができます.
定期的な再生とクリーニング
定期的なメンテナンスは、非活性化触媒の活性を回復させます。 化学薬品の洗うことか超音波処置のようなクリーニング方法は、汚れおよび表面の汚染物を取り除きます。 熱または電気化学的処置を含む再生技術は、失われた活動的な場所を回復します。 メンテナンススケジュールの実装により、触媒は長期にわたって効率的なままになります.
腐食抵抗触媒の開発
耐腐食性触媒は、従来の材料よりも優れた過酷な環境に耐える。 材料科学の進歩は改善された化学安定性の触媒の作成に導きました。 例えば、非金属元素を触媒構造に組み込むことで、酸化や剥離に対する耐性が向上します。 これらの革新は頻繁に取り替えのための必要性を減らす電解槽触媒の寿命を増加します.
コールアウト 耐腐食性材料への投資は、運用コストを削減し、システム信頼性を向上させます.
濾過、中毒、熱分解などのメカニズムから電解触媒の非活性化が生じる。 分光や電気化学試験などの診断技術は、これらの問題に重要な洞察を提供します。 防蝕材料および保護コーティングの進歩は約束を示します。 将来の研究は、産業水素生産のための触媒耐久性を高めるためのスケーラブルソリューションに焦点を当てるべきです.
よくあるご質問
水電解中の触媒活性の最も一般的な原因は何ですか?
ファーリングと表面ブロックは、最も頻繁な原因です。 不純物および反応副産物は触媒に蓄積し、活動的な表面区域および効率を減らします.
オペレータが触媒劣化の早期徴候を検出する方法は?
オペレータは性能を監察するために周期的なvoltammetryのような電気化学テストを、使用することができます。 これらの方法は減らされた活動か高められた抵抗を、潜在的な低下を信号で識別します.
従来よりも高価な耐食性触媒はありますか?
耐腐食性触媒は、多くの場合、より高い初期コストを持っています。 しかし、それらの延長寿命および減らされた維持の条件はそれらを作ります 長期適用のための費用効果が大きい.